How does an electron microprobe work?
In an electron microprobe, a solid sample is bombarded with a focused beam of high energy electrons (5 - 30 keV) which results in a variety of different types of interactions between the beam electrons and the atoms in the sample. Several of these interactions produce signals that can be detected and used to provide information about the nature of the material being examined. For the purposes of electron probe microanalysis (EPMA), the most important of these interactions is when a beam electron collides with an atom in the sample and causes an inner shell electron to be ejected from the atom. The inner shell vacancy leaves the atom in an high-energy excited state, and it loses this excess energy by either 1) having an outer shell electron move to fill the inner shell vacancy, accompanied by the release of a x-ray photon or 2) the ejection of a outer shell (auger) electron. An x-ray photon produced by the first process has an energy equal to the difference in energy between the two shells involved in the transition. For a given transition between a specific outer and inner shell, the energy of the emitted x-ray photon will uniformly increase with increasing atomic number (Z) of the excited atom. The reason is that the increase in the positive charge of the nucleus with atomic number causes the electrons in all shells to be bound more tightly and thus have higher energy as Z increases. As a result, for a given transition, each element will produce x-rays with a unique (characteristic) energy (or wavelength). It is these "characteristic x-ray lines", ranging in energy from 0.1 - 15 keV that the electron microprobe uses to identify and quantify the elements present in the sample.
X-ray lines and Quantitative Analysis
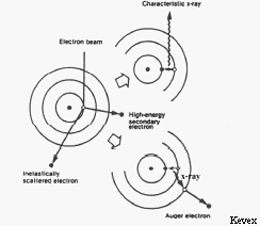
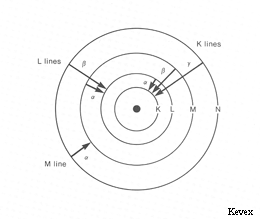
In x-ray notation, the electron shells of an atom are labeled K, L, M, N, and O starting with the innermost (lowest energy) shell. These shells correspond to orbitals with principal quantum numbers n=1,2,3... (i.e. s,p,d,f,... in quantum notation). The transitions giving rise to the x-ray lines most often used in electron microprobe analysis are the transitions Liii - K (the Kα line), Mv - Liii (the Lα line), and Nvii - Mv (the Mα line) (i.e. in quantum notation 2p3/2 - 1s1/2, 3d5/2 - 3p3/2, and 4f7/2 - 3d5/2 respectively).
The x-rays produced by the above process are detected and counted by several (usually 4-6) wavelength dispersive (WDS) spectrometers arrayed around the sample chamber. Each spectrometer contains a crystal which can be positioned at an angle (as determined by Bragg's Law) to reflect (actually diffract) a specific x-ray wavelength coming from the sample into an x-ray counter while allowing the other wavelengths to be absorbed by the crystal. The rate at which x-ray photons of a given element (Mg for example) are produced by the sample can be determined by setting the crystal to the position which will reflect Mg x-rays into the detector and then counting the number of x-ray photons detected over a specified length of time. As the crystal of a WDS spectrometer can only collect x-ray counts for one wavelength at a time, the analysis of multiple elements on a single spectrometer must be done sequentially. In addition, no single type of crystal can satisfy Braggs Law for the full range of x-ray wavelengths emitted by all the elements in the periodic table. For these reasons, most modern electron microprobes are equipped more than one spectrometer and also the ability to automatically exchange different crystals within a single spectrometer. For example, our microprobe has 4 WDS spectrometers containing 12 diffracting crystals which allows us to collect x-ray counts on four elements simultaneously for all elements Z ≥ 4.
Quantitative electron probe microanalysis is a standards-based technique. In order to turn the x-ray count rates obtained for an element in an unknown sample into a concentration (in wt%) it is necessary to compare the unknown count rate to the count rate obtained on a standard with a known composition under the same analytical conditions. For example, if under the same conditions you get 20 counts/sec on an unknown and 40 counts/sec on a standard containing 50 wt% Mg, to a first approximation (known as Castaing's First Approximation) the unknown is calculated to contain (20/40) * 50 wt% Mg = 25 wt% Mg. In practice, this approximation must be adjusted to account for the effects of other elements present in the sample and standard that may differentially affect x-ray production for the element of interest (i.e. matrix effects) in order to arrive a final estimate of the concentration of elements present in the unknown.
Other Useful Signals
Backscattered Electrons (BSE)
Besides characteristic x-rays, electron beam-sample interactions produce a number of other useful signals that can be detected by the electron microprobe. One of the most useful of these interactions is the production of backscattered electrons (BSE). A backscattered electron is a beam electron whose trajectory has been deflected so much by coulombic interactions with atoms in the sample (i.e. by elastic scattering events) that it is turned around and remerges from the sample surface with relatively high energy. The number of beam electrons that are backscattered is strongly dependent on the average atomic number (Z) of the atoms in the sample. By placing backscattered electron detectors in the roof of the sample chamber and rastering the electron beam over the sample surface it is possible to produce a composition map showing areas of the sample having different average atomic numbers as different shades of gray. BSE images of this type are extremely useful in analyzing multiphase samples.
Secondary Electrons (SE)
Interactions in which energy is transferred from beam electrons to atoms in the sample (i.e. inelastic scattering events) can cause loosely bound outer electrons of the sample atoms to gain enough energy that they can move through the sample. These secondary electrons (SE) have such low energy that only those produced very near the surface can escape the sample and be detected. As a result, secondary electrons can be used to produce high-resolution images of the sample surface that are used in electron microscopy.
Cathodoluminescence (CL)
Some insulators and semi-conductors produce UV to near-IR radiation (including visible light) when bombarded by the electron beam, due to the excitation and decay of electron-hole pairs in the target atom. The color and intensity of this radiation is extremely sensitive to trace element content and lattice defects. Images of this radiation can be very useful in studies growth zoning in minerals and other materials. Our lab currently does not have the equipment needed to detect these signals. However CL imaging can be performed using SEMs in the geosciences department.
Other Not So Useful Signals
Background Radiation (Bremsstrahlung)
Beam electrons can undergo deceleration when they encounter the coulombic field of atoms in the sample. The energy lost as the beam electron slows down is emitted as electromagnetic radiation that can range in energy from zero up to the full energy of the beam electron. X-rays produced by this process form a continuous background spectrum in the electron microprobe which needs to be accounted for when attempting to measure the intensity of characteristic x-ray lines. This is usually done by measuring x-ray intensity at both higher and lower wavelengths than that of the x-ray line of interest, using these to interpolate the amount of background radiation at the desired wavelength, and subtracting this background from the total measured x-ray counts to determine the true intensity of the characteristic x-ray line of interest.
Heat
A large fraction of the energy of the electron beam ultimately excites lattice vibration in the sample and thus produces heat. Specimen heating by the electron beam can sometimes cause "beam damage" to occur in the sample, especially if the sample contains volatile elements, has a low melting temperature, or has a low average atomic number.